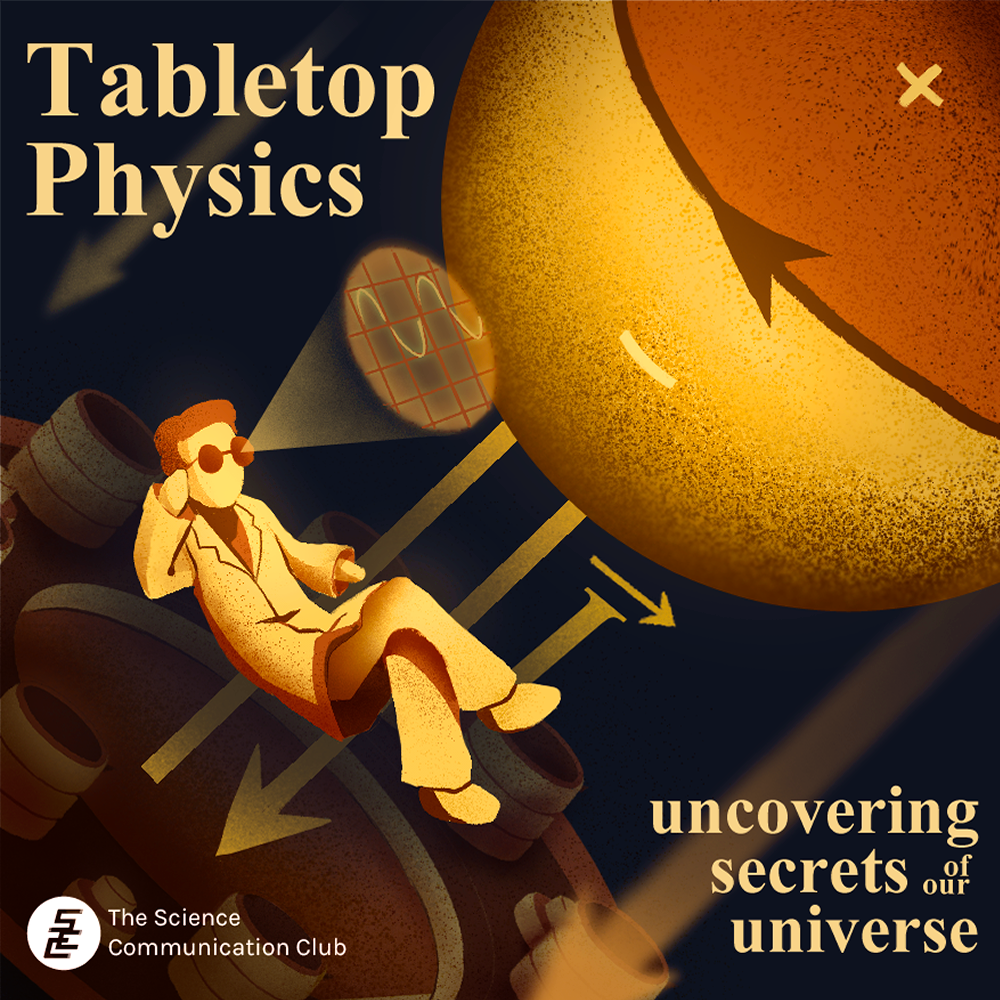
Written by Harish Ramachandran
Illustrated by Yuki Lee
Many of the most awe-inspiring unsolved problems in physics deal with extremes of our universe —from the happenings of subatomic particles to those at the centres of black holes. Some of them tackle whether we can reconcile our existing understandings of these disparate regimes. Could we someday have a unifying theory of the physics of both the smallest and largest scales in the universe? In some cases, the practical approaches to conducting that search are starting to form their own dichotomy: between brute force and finesse.
In the modern era, the lauded and newsworthy experimental discoveries in these areas of physics have frequently been borne of “brute force”. This approach consists of large collaborations of scientists collecting vast amounts of data from physically massive experiments. For instance, the 2013 Nobel Prize in Physics was awarded for the theoretical discovery of the Higgs boson: a fundamental particle posited in 1964.1 Its existence had recently been validated by experiments at the Large Hadron Collider — a 27 km-long circular tunnel — conducted by almost 6,000 scientists. In 2017, the Prize was awarded for the observation of gravitational waves by the Laser Interferometer Gravitational-Wave Observatory.2 This involved over 1,000 scientists and a pair of four km-long tunnels. Then, in 2020, the Prize was awarded for the prediction (1965) and subsequent imaging of a black hole (2019).3 To generate the photo, over 300 scientists synchronized radio telescopes separated by hundreds of thousands of kilometers around the globe. Many would argue that such collaborations are the future of particle and astrophysics.
It turns out, however, that by performing very precise measurements on atoms and molecules cooled to near absolute zero temperature, physicists can unveil this collider-scale new physics in clever, tabletop-sized experiments. Herein lies the “finesse” approach. Although the effects of high energy physics or cosmology on a mere atom are tiny, the trick is that these systems can be controlled extraordinarily well and reproduced identically many, many times. The instrumentation (e.g. lasers and electronics) underpinning the research has quickly become very stable and reliable, owing to diverse commercial interests.4 As a result, the statistical precision on these experiments is often limited only by measurement uncertainties inherent to microscopic physics (i.e. due to quantum mechanics5) rather than the poor resolution of the equipment.
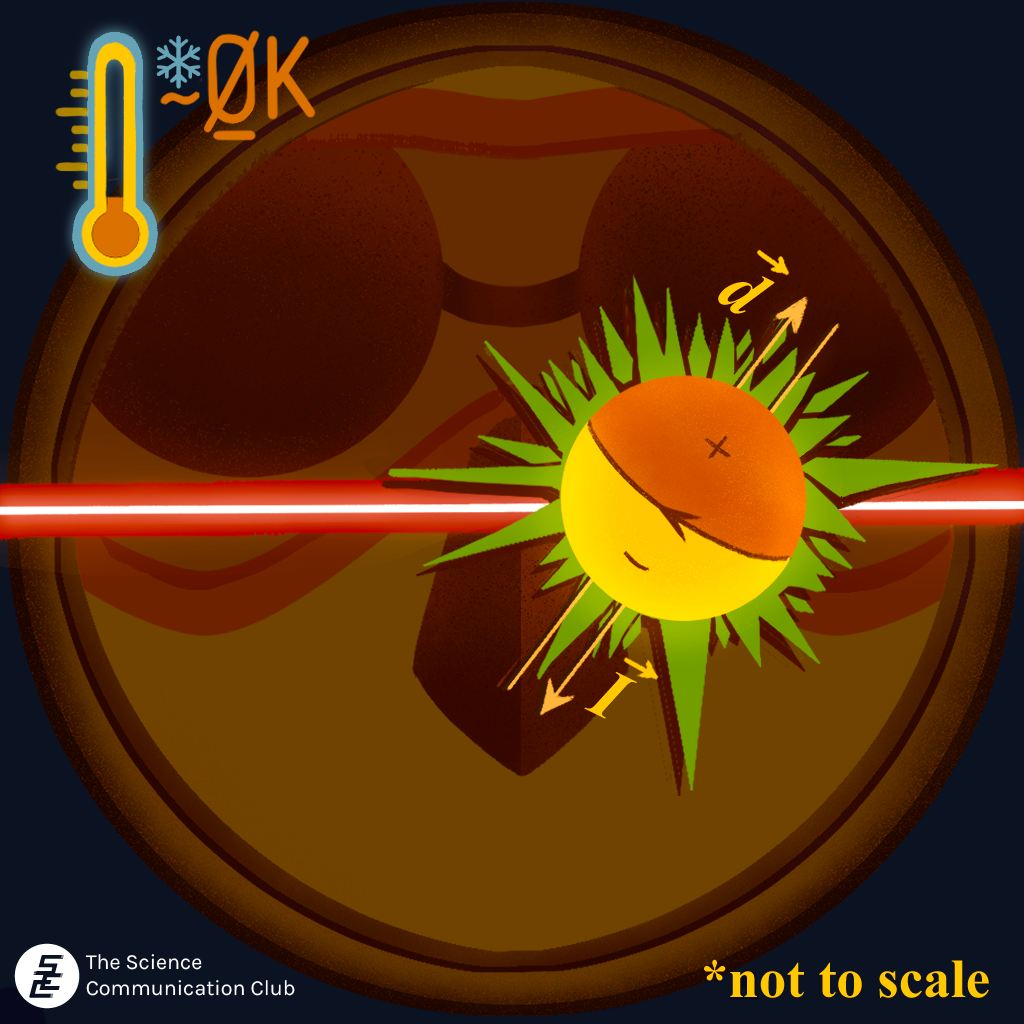
To distinguish new physics from other spurious signals, however, the systems also must be very well-understood theoretically. Fortunately, some atoms are simple enough to satisfy this criterion. Beyond that, successful experiments tend to involve clever measurement schemes and sequences to cancel out all spurious effects. Any slight discrepancies between experiment and theory then present signals of undiscovered physics.6 Altogether, these experiments have a certain charm: a few grad students toiling away at a small table in a basement somewhere may very well glimpse the deepest secrets of our universe.
Tabletop precision measurements can help answer a few far-reaching questions. In particular, according to astronomical observations, our universe is largely made up of stuff we do not understand. Only about 5% of it is matter: all the particles we know about, which form the basis for everything we see all around us. The majority (95%) is stuff that physicists know must be out there but have no idea of what it is.7 This stuff — called dark matter and dark energy — must exist because its fingerprints are on our universe. It’s why our universe is expanding7 and why galaxies rotate faster than we expect. Physicists have theories for what dark matter and energy could be and are looking for it, but none of them yet have convincing evidence. Even for the 5% we purportedly understand, we do not know how and why our universe stabilized with that much matter.
Atomic experiments turn out to be incredibly sensitive to the potential new physics behind the structure and composition of our universe. They are equivalent to searches in colliders with approximately 100 times more energy than the Large Hadron Collider!8 It turns out that these measurements are great at answering questions about dark matter9, dark energy10, and even unifying theories resolving inconsistencies between theories in physics.11 With how powerful these tabletop experiments have become, they could be the source of our next major breakthroughs in our fundamental understanding of the universe.
Sources:
- Scientific Background on the Nobel Prize in Physics 2013 [online document]. NobelPrize.org. 2013 Oct 8 [accessed 2022 Sep 15]. https://www.nobelprize.org/prizes/physics/2013/advanced-information/
- Scientific Background on the Nobel Prize in Physics 2017 [online document]. NobelPrize.org. 2017 Oct 3 [accessed 2022 Sep 15]. https://www.nobelprize.org/prizes/physics/2017/advanced-information/
- Scientific Background on the Nobel Prize in Physics 2020 [online document]. NobelPrize.org. 2020 Oct 6 [accessed 2022 Sep 15]. https://www.nobelprize.org/prizes/physics/2020/advanced-information/
- Arrigoni M, Vogt P. Industrial Reliability in Scientific Lasers. Optik & Photonik. 2016;Vol.11(1): p. 20-23. doi: 10.1002/opph.201600003
- Jaekel MT, Reynaud S. Quantum limits in interferometric measurements. EPL. 1990;Vol.13(4): p. 301-306. doi: 10.1209/0295-5075/13/4/003
- Baron J, Campbell WC, DeMille D, Doyle JM, Gabrielse G, Gurevich YV, Hess PW, Hutzler NR, Kirilov E, Kozyryev I, et al. Methods, analysis, and the treatment of systematic errors for the electron electric dipole moment search in thorium monoxide. New J. Phys. 2017;Vol.19(7): 073029. doi: 10.1088/1367-2630/aa708e
- North C. Cosmic Microwave Background [blog]. Planck Satellite. 2013 Mar 21 [accessed 2022 Sep 15]. https://plancksatellite.org.uk/results/cosmic-microwave-background/
- Ibrahim T, Itani A, Nath P. Electron electric dipole moment as a sensitive probe of PeV scale physics. Phys. Rev. D. 2014;Vol.90(5): 055006. doi: 10.1103/physrevd.90.055006
- Van Tilburg K, Leefer N, Bougas L, Budker D. Search for ultralight scalar dark matter with atomic spectroscopy. Phys. Rev. Lett. 2015;Vol.115(1): 0118002. doi: 10.1103/physrevlett.115.011802
- Elder B, Khoury J, Haslinger P, Jaffe M, Müller H, Hamilton P. Chameleon Dark Energy and atom interferometry. Phys. Rev. D. 2016;Vol.94(4): 044051. doi: 10.1103/physrevd.94.044051
- Becker A. One lab’s quest to build space-time out of quantum particles [online article]. Quanta Magazine. 2021 Nov 24 [accessed 2022 Sep 15]. https://www.quantamagazine.org/one-labs-quest-to-build-space-time-out-of-quantum-particles-20210907/